All stars form in nebulae, huge clouds of gas and dust.
Though they shine for thousands of years, stars do not last forever. The changes that occur in a star over time and the final stage of its life depends on a star's size.
The exact lifetime of a star depends very much on its size. Very massive stars use up their fuel quickly. This means they may only last a few hundred thousand years.
Smaller stars use fuel more slowly and will shine for several billion years.
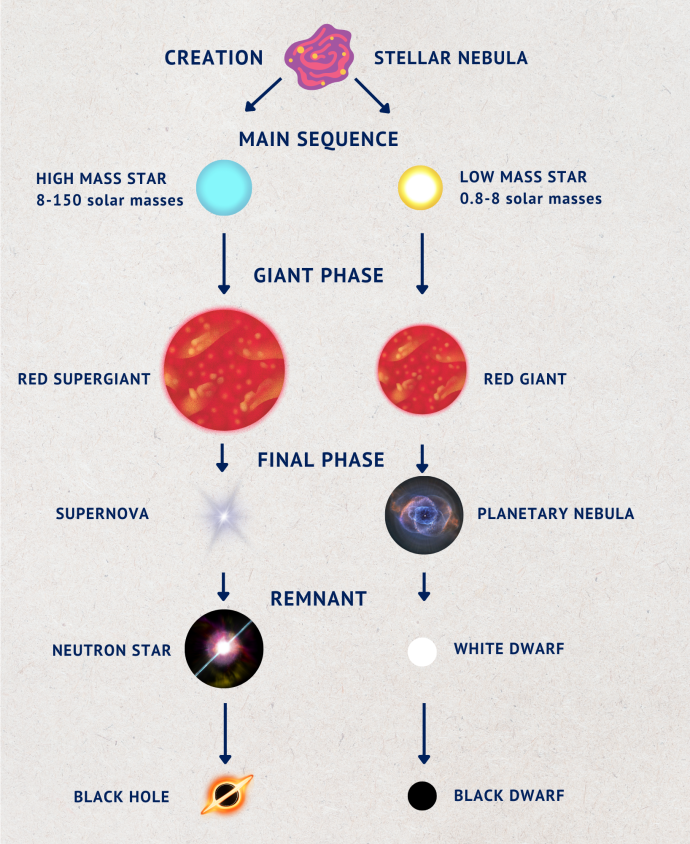
Eventually, the hydrogen that powers a star's nuclear reactions begins to run out. The star then enters the final phases of its lifetime. All stars will expand, cool and change colour to become a red giant or red supergiant.
What happens next depends on how massive the star is.
A smaller star, like the Sun, will gradually cool down and stop glowing. During these changes, it will go through the planetary nebula phase and white dwarf phase. After many thousands of millions of years, it will stop glowing and become a black dwarf.
A massive star experiences a much more energetic and violent end. It explodes as a supernova. This scatters materials from inside the star across space. This material can be collected in nebulae and form the next generation of stars.
After the dust clears, a very dense neutron star is left behind. These spin rapidly and can give off streams of radiation, known as pulsars. If the star is especially massive, it forms a black hole when it explodes.
You can read more about each of these stages below.
- Main Sequence
Nuclear reactions at a star's centre (or core) provide the energy that make sit shine brightly. This stage is called the 'main sequence'.
Main-sequence stars are fusing hydrogen into helium. This nuclear fusion take place deep in the cores of stars. Stars spend about 90% of their lives in this stage. Our Sun is about 5,000 million years into its 10,000 million-year main-sequence.
One of the fundamental forces in the Universe is gravity. It is the force that holds us on Earth. It also keeps planets in orbit around stars and stars in orbit around the centre of our galaxy.
A star's gravity pulls in on itself. This should cause a star to collapse. Unlike the Earth, stars are not solid. So what prevents them from collapsing? In main-sequence stars, it is the pressure from the nuclear fusion reactions deep in the star's core. This balance between outward pressure and inward pull is called hydrostatic equilibrium.
ImageCreditThis work by The Schools' Observatory is licensed under All rights reservedThe outward force by fusion (red arrows) is balanced by the inwards force from gravity (blue arrows). Note how all the arrows are the same size. It may seem unlikely that the forces balance so neatly, but they actually work very well together. If something happens to reduce the amount of pressure generated by the star's core, the star begins to contract. This contraction increases the temperature and pressure which allows the core to release more energy. A new balance is found and hydrostatic equilibrium is maintained. In addition, the star now has a slightly smaller radius.
The mass of a star controls how long it stays in the main-sequence stage. More massive stars use up their fuel more rapidly than less massive ones. When a star runs out of fuel, it can no longer keep the forces in balance. At this point, a star expands and cools to become either a red giant or a red supergiant star.
The next stages (after the main sequence) for low-mass stars:
- Red Giant
Stars spend most of their lives in the main-sequence stage. When the hydrogen in the centre of a star runs out, the star begins to use hydrogen further out from its core.
This causes it to start to grow. Its radius can reach up to 400 times its original size. As the star expands it also cools. The change in temperature causes the star to glow redder. The star is now a red giant.
Red giants can be 20 and 100 times the size of the Sun though only contain 0.25 to 8 times the mass of the Sun. They are also very bright stars. The surface temperature of a red giant is less than 4,000 - 5,000 K.
ImageCreditThis work by Oona Räisänen is licensed under Creative Commons Attribution Share Alike 4.0 InternationalSize comparison of the Sun as a main-sequence star and the Sun as a red giant Over time, as the outer layers of the star expand, gravity causes its core to shrink and contract. The temperature and pressure in the centre increase until nuclear fusion can start again. Now the core is fusing helium, rather than hydrogen.
The star, now powered by helium, starts to shrink, get hotter and turn blue. However, the star's supply of helium quickly runs out, so this stage only lasts for about a million years. When the helium runs out, the core shrinks again. This time the star begins to use helium further out from its core. At the same time, it may start fusing hydrogen in a shell around the helium fusion!
The outer layers of the star expand, cool and turn red again. It has entered its second red giant phase.
Red giants can swallow up planets as they expand. The Sun will reach its red giant stage in about 5,000 million years time. During this phase, it will probably engulf the inner planets of our Solar System which could include the Earth. But don't worry! This won't happen for a very long time.
- Planetary Nebula
The planetary nebula phase is a final stage in a low-mass star's life. During this phase, the star sheds its outer layers. This creates an expanding, glowing shell of very hot gas.
Despite the name, they have nothing to do with planets. They got this name because astronomers using small telescopes long ago, and they thought they looked a bit like planets.
ImageCreditThis work by NASA/HST is licensed under Creative Commons Zero v1.0 UniversalA planetary nebula (Helix Nebula) Low-mass stars turn into planetary nebulae towards the end of their red giant phase. At that point, the star becomes highly unstable and starts to pulsate. This produces strong stellar winds, which throw off the outer layers of the star.
The outer layers drift away from the star, leaving a small, hot, bright core behind, called a white dwarf. The white dwarf gives of ultraviolet radiation which lights up the layers of gas around the star.
Over time, the material from the planetary nebula is scattered into space. Eventually it will form part of the clouds of dust and gas where new stars form.
Planetary nebula last just a few tens of thousands of years. This is short compared to the thousands of millions of years which low-mass stars shine for.
Our Sun is a low-mass star and will produce a planetary nebula in about 5,000 million years time.
- White Dwarf
About 6 % of all known stars in our part of the Milky Way are white dwarfs. When a small star runs out of fuel, it produces a planetary nebula. The outer layers of the nebula drift away from the star, leaving a white dwarf.
A white dwarf is a bright, hot, compact star. They are about the same size, in terms of volume, as the Earth. However, they contain about as much mass as the Sun.
ImageCreditThis work by NASA/S.Charbinet is licensed under Creative Commons Zero v1.0 UniversalWhite dwarf in comparison to Earth Because of their small radius, they are very faint. A typical white dwarf shines with only 0.1& - 1 % of the brightness of the Sun. Our nearest white dwarf is Sirius B, but it is too faint to see with an optical telescope.
White dwarfs do not release energy through nuclear fusion reactions. The light and heat they emit are left over from previous stages of their evolution. Despite this, white dwarfs have some of the hottest surface temperatures of any star. They can be over 100,000 °C!
The material within a white dwarf was created by its parent star during its main sequence and red giant phases. This material is compacted into a relatively small space, which makes white dwarfs very dense. The density of a white dwarf is about 1 million tonnes per cubic metre which is 200,000 times as dense as the Earth! Imagine the mass of the Sun, squashed to the size of the Earth! A matchbox of white dwarf material would weigh the same as fifteen elephants!
The material is so compact it reaches a state known as neutron degeneracy. The normal relationships between temperature, pressure and density do not hold for degenerate matter. As the mass of a white dwarf increases, its radius decreases. There is a maximum mass beyond which a white dwarf becomes unstable and collapses to form a black hole. This limit (known as the Chandrasekhar Limit) is about 1.44 solar masses.
- Black Dwarf
White dwarfs do cool down, but very slowly. Their small surface area means they radiate their heat away very slowly. It will take a white dwarf billions of years to cool down to temperatures near 10,000 K. For comparison, the Sun's surface temperature is around 6,000 K.
A black dwarf is just a white dwarf that has cooled downed so much that it no longer emits any heat or light. The time it takes for this to happen is longer than the current age of the universe (13.8 billions years), so no black dwarfs exist yet. This makes them theoretical objects, not real ones.
The next stages (after the main sequence) for high-mass stars:
- Red Supergiant
Supergiants have the largest radius of all known stars. They evolve from main-sequence stars. Supergiants contain 8 - 200 times the mass of the Sun! They also shine brightly. One supergiant can be as bright as a million Suns.
ImageCreditThis work by Rogelio Bernal Andreo is licensed under Creative Commons Attribution Share Alike 4.0 InternationalThe Orion region showing the red supergiant Betelgeuse in the upper left corner Supergiants use up the hydrogen and helium in their cores within a few million years. They then start to burn carbon, and the stars expands and cools. Red supergiants have low surface temperatures below 4, 100 K.
This continues with heavier and heavier elements until it contains a core of iron. At this point, fusion stops, and the star collapses under its own gravity, creating a supernova explosion.
- Supernova
Supernova are huge explosions in space. They take place during the final stages of a some stars' lives. When they erupt, one supernova can briefly shine brighter than a whole galaxy.
There are different types of supernovae.
Core-collapse Supernovae
The most well known are core-collapse supernovae. These take place when a star, at least 8 times the mass of the Sun, runs out of fuel.
When a massive star reaches the final stages of its evolution, its core is made mostly of iron. The star cannot fuse elements heavier than iron. This means fusion stops. At this point, there is no outward pressure to balance the inward pull of its gravity. Gravity pulls all the material in the star towards its middle. This starts a sudden, rapid collapse of the star.
The outer layers of the star collapse inwards until they reach the core. They bounce off the surface of the dense iron core at around 30, 000 kilometres per second. This sends shock waves through the star. The shock waves cause the star to explode as a supernova.
Huge amounts of energy are created during the collapse, and new elements form in the process. The star brightens quickly, then gradually fades away, leaving only core. During the explosion, the core collapses down to create either a neutron star or a black hole.
The material which was thrown out during the explosion surrounds the core. We call this a supernova remnant. It will eventually drift into space and become part of a huge cloud of dust and gas. New stars will form in this cloud. This is how elements made in the first stars were recycled to make everything in our Solar System, including us!
ImageCreditThis work by NASA/ESA/J. Hester/A. Loll (Arizona State University) is licensed under Creative Commons Zero v1.0 UniversalThe Crab Nebula, a supernova remnant in our Milky Way galaxy. The supernova happened in the year 1054, and was so bright that it was visible in the sky during daytime for almost an entire month! Type Ia Supernovae
The other type of supernova are called Type Ia, or thermonuclear explosions. This type of explosion does not occur when a massive star's core collapses. They instead happen in a binary (or double) star system. To trigger a type Ia supernova, one of two stars must be a white dwarf. The other star is often a low-mass star, like our Sun, or can be red giant star.
Because it is so dense, the surface of a white dwarf has very strong gravity. This gravity pulls material from the nearby star onto the white dwarf, and as it pulls in material from its companion star, the white dwarf becomes more and more massive. This process is known as accretion.
Something called electron degeneracy pressure stops the white dwarf from collapsing. This means the electrons inside each atom repel each other. This prevents the white dwarf from shrinking any further.
During accretion, the mass of the white dwarf increases. If the white dwarf grows to over 1.44 times the mass of the Sun, the electrons are no longer strong enough to prevent the star from collapsing. At this point, the star explodes as a type Ia supernova. This mass limit is called the Chandrasekhar limit. During this type of explosion, the star is destroyed.
Type Ia supernovae are one of the brightest events in the Universe. They are many times brighter other kinds of supernovae.
ImageCreditThis work by Las Cumbres Observatory/PTF/B.J. Fulton is licensed under Creative Commons Attribution Non Commercial 4.0 InternationalThe galaxy M101 before and after supernova SN 2011fe exploded Type Ia supernovae always have the same brightness. This is because the explosion always takes place when the white dwarf reaches a set mass. We call objects of known brightness 'standard candles'.
We use standard candles - like type Ia explosions - to measure distances in space. These observations have helped prove the Universe is expanding and accelerating.
- Neutron Stars
A neutron star is the collapsed core of a massive star. It is what is left of the star, after a supernova explosion.
When a high-mass star comes to the end of its lifetime, its outer layers collapse onto the core. This squashes the star's core to the point where the atoms are smashed to pieces, leaving only neutrons. Neutrons are sub-atomic particles with no electric charge.
A neutron star can have the same mass as 1 or 2 Suns. However, it will only be about 20 km across. Imagine squashing the Sun until it was the size of a city! For comparison, a house full of neutron star material would weigh the same as the Moon. The only object denser than a neutron star is a black hole.
ImageCreditThis work by NASA/Casey Reed - Penn State University is licensed under Creative Commons Zero v1.0 UniversalArtist's impression of a neutron star Some neutron stars have been found to rotate several hundred times a second! These rotating neutron stars are called Pulsars.
Pulsars emit beams of radio waves and other radiation. We can only detect them if the beam points towards Earth, so there may more be pulsars that we cannot observe. As the neutron star spins, the beams sweep past the Earth at regular intervals, or in pulses, hence the name. It's a bit like seeing the flashes of light from a lighthouse.
The amount of time between each pulse can be as long as 20 seconds and as short as a few thousandths of a second. This is incredibly fast when compared to the 24 hours it takes the Earth to rotate.
The first pulsar was discovered in 1967 by Jocelyn Bell Burnell and Antony Hewish. At first, they thought this regularly repeating radio signal could be from aliens, so they named it LGM-1 (Little Green Men 1). But they soon realized that this was not the case!
- Black Holes
Black holes are very strange objects. They are made during supernova explosions. These take place when very massive stars come to the end of their lives.
After the supernova, anything left of the star is squashed and compacted into an incredibly small, dense object. This is the black hole. Once a black hole has formed, it grows by pulling in gas, dust, stars, and even other black holes around it.
In 2015, scientists first detected gravitational waves. These ripples in space-time were caused by two black holes colliding and shaking the Universe.
- Gamma Ray Bursts
Gamma-ray bursts (or GRBs) are very short and intense bursts of gamma-ray radiation. If we take a look at the electromagnetic spectrum, we can see that gamma rays are even higher in energy than X-rays. This makes the events which create them extremely high-energy events.
GRBs were first discovered in 1967 by the Vela satellites. These satellites were not designed to search for high energy events in space. They were designed to detect Soviet nuclear missile tests which breached the nuclear test ban treaty. Their gamma radiation detectors - meant to detect radiation from nuclear missiles on Earth - instead picked up gamma-ray bursts coming from outside our Solar System!
Not all GRBs are the same. GRBs can last for different amounts of time, ranging from thousandths of a second to minutes. The amount of energy they emit also varies. A key focus of current GRB research is to investigate what creates the different types of GRBs.
Short gamma-ray bursts
GRBs which last for less than 2 seconds are short gamma-ray bursts. The mean average duration of short GRBs is 0.2 seconds. This type of GRB makes up about 30 % of the total number observed.
They have been linked to high-energy collision events. For example mergers between black holes or neutron stars. These type of objects have very strong magnetic fields. The fields eject the GRBs as jets from the object's magnetic field poles.
ImageCreditThis work by NASA/Swift/Mary Pat Hrybyk-Keith and John Jones is licensed under Creative Commons Zero v1.0 UniversalArtist's illustration of a bright GRB Long gamma-ray bursts
GRBs which last for more than 2 seconds are long gamma-ray bursts. This type of GRB makes up about 70 % of the total number observed.
We have been able to investigate this kind of GRB in more detail than short GRBs. This is because they last longer, there are more of them, and they have brighter 'afterglows'.
This type of GRBs has been linked to the deaths of massive stars in galaxies where stars form very rapidly.